Admin مدير المنتدى


عدد المساهمات : 18726 التقييم : 34712 تاريخ التسجيل : 01/07/2009 الدولة : مصر العمل : مدير منتدى هندسة الإنتاج والتصميم الميكانيكى
 | موضوع: كتاب Small Unmanned Fixed-Wing Aircraft Design - A Practical Approach الخميس 07 سبتمبر 2023, 1:40 am | |
| 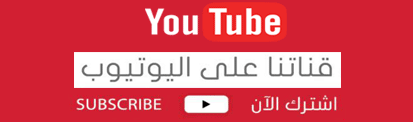
أخواني في الله أحضرت لكم كتاب Small Unmanned Fixed-Wing Aircraft Design - A Practical Approach Andrew J. Keane University of Southampton UK András Sóbester University of Southampton UK James P. Scanlan University of Southampton UK
 و المحتوى كما يلي :
Contents List of Figures xvii List of Tables xxxiii Foreword xxxv Series Preface xxxvii Preface xxxix Acknowledgments xli PART I INTRODUCING FIXED-WING UAVS 1 Preliminaries 3 1.1 Externally Sourced Components 4 1.2 Manufacturing Methods 5 1.3 Project DECODE 6 1.4 The Stages of Design 6 1.4.1 Concept Design 8 1.4.2 Preliminary Design 10 1.4.3 Detail Design 11 1.4.4 Manufacturing Design 12 1.4.5 In-service Design and Decommissioning 13 1.5 Summary 13 2 Unmanned Air Vehicles 15 2.1 A Brief Taxonomy of UAVs 15 2.2 The Morphology of a UAV 19 2.2.1 Lifting Surfaces 21 2.2.2 Control Surfaces 22 2.2.3 Fuselage and Internal Structure 23 2.2.4 Propulsion Systems 24x Contents 2.2.5 Fuel Tanks 24 2.2.6 Control Systems 24 2.2.7 Payloads 27 2.2.8 Take-off and Landing Gear 27 2.3 Main Design Drivers 29 PART II THE AIRCRAFT IN MORE DETAIL 3 Wings 33 3.1 Simple Wing Theory and Aerodynamic Shape 33 3.2 Spars 37 3.3 Covers 37 3.4 Ribs 38 3.5 Fuselage Attachments 38 3.6 Ailerons/Roll Control 40 3.7 Flaps 41 3.8 Wing Tips 42 3.9 Wing-housed Retractable Undercarriage 42 3.10 Integral Fuel Tanks 44 4 Fuselages and Tails (Empennage) 45 4.1 Main Fuselage/Nacelle Structure 45 4.2 Wing Attachment 47 4.3 Engine and Motor Mountings 48 4.4 Avionics Trays 50 4.5 Payloads – Camera Mountings 51 4.6 Integral Fuel Tanks 52 4.7 Assembly Mechanisms and Access Hatches 54 4.8 Undercarriage Attachment 55 4.9 Tails (Empennage) 57 5 Propulsion 59 5.1 Liquid-Fueled IC Engines 59 5.1.1 Glow-plug IC Engines 62 5.1.2 Spark Ignition Gasoline IC Engines 62 5.1.3 IC Engine Testing 65 5.2 Rare-earth Brushless Electric Motors 66 5.3 Propellers 68 5.4 Engine/Motor Control 70 5.5 Fuel Systems 70 5.6 Batteries and Generators 71 6 Airframe Avionics and Systems 73 6.1 Primary Control Transmitter and Receivers 73 6.2 Avionics Power Supplies 76Contents xi 6.3 Servos 78 6.4 Wiring, Buses, and Boards 82 6.5 Autopilots 86 6.6 Payload Communications Systems 87 6.7 Ancillaries 88 6.8 Resilience and Redundancy 90 7 Undercarriages 93 7.1 Wheels 93 7.2 Suspension 95 7.3 Steering 95 7.4 Retractable Systems 97 PART III DESIGNING UAVS 8 The Process of Design 101 8.1 Goals and Constraints 101 8.2 Airworthiness 103 8.3 Likely Failure Modes 104 8.3.1 Aerodynamic and Stability Failure 105 8.3.2 Structural Failure 106 8.3.3 Engine/Motor Failure 107 8.3.4 Control System Failure 107 8.4 Systems Engineering 110 8.4.1 Work-breakdown Structure 110 8.4.2 Interface Deinitions 112 8.4.3 Allocation of Responsibility 112 8.4.4 Requirements Flowdown 112 8.4.5 Compliance Testing 113 8.4.6 Cost and Weight Management 114 8.4.7 Design “Checklist” 117 9 Tool Selection 119 9.1 Geometry/CAD Codes 120 9.2 Concept Design 123 9.3 Operational Simulation and Mission Planning 125 9.4 Aerodynamic and Structural Analysis Codes 125 9.5 Design and Decision Viewing 125 9.6 Supporting Databases 126 10 Concept Design: Initial Constraint Analysis 127 10.1 The Design Brief 127 10.1.1 Drawing up a Good Design Brief 127 10.1.2 Environment and Mission 128 10.1.3 Constraints 129xii Contents 10.2 Airframe Topology 130 10.2.1 Unmanned versus Manned – Rethinking Topology 130 10.2.2 Searching the Space of Topologies 133 10.2.3 Systematic “invention” of UAV Concepts 136 10.2.4 Managing the Concept Design Process 144 10.3 Airframe and Powerplant Scaling via Constraint Analysis 144 10.3.1 The Role of Constraint Analysis 144 10.3.2 The Impact of Customer Requirements 145 10.3.3 Concept Constraint Analysis – A Proposed Computational Implementation 145 10.3.4 The Constraint Space 146 10.4 A Parametric Constraint Analysis Report 146 10.4.1 About This Document 146 10.4.2 Design Brief 147 10.4.3 Unit Conversions 149 10.4.4 Basic Geometry and Initial Guesses 151 10.4.5 Preamble 151 10.4.6 Preliminary Calculations 152 10.4.7 Constraints 154 10.5 The Combined Constraint Diagram and Its Place in the Design Process 162 11 Spreadsheet-Based Concept Design and Examples 165 11.1 Concept Design Algorithm 166 11.2 Range 169 11.3 Structural Loading Calculations 169 11.4 Weight and CoG Estimation 170 11.5 Longitudinal Stability 170 11.6 Powering and Propeller Sizing 171 11.7 Resulting Design: Decode-1 174 11.8 A Bigger Single Engine Design: Decode-2 177 11.9 A Twin Tractor Design: SPOTTER 182 12 Preliminary Geometry Design 189 12.1 Preliminary Airframe Geometry and CAD 190 12.2 Designing Decode-1 with AirCONICS 192 13 Preliminary Aerodynamic and Stability Analysis 195 13.1 Panel Method Solvers – XFoil and XFLR5 196 13.2 RANS Solvers – Fluent 200 13.2.1 Meshing, Turbulence Model Choice, and y+ 204 13.3 Example Two-dimensional Airfoil Analysis 208 13.4 Example Three-dimensional Airfoil Analysis 210 13.5 3D Models of Simple Wings 212 13.6 Example Airframe Aerodynamics 214 13.6.1 Analyzing Decode-1 with XFLR5: Aerodynamics 215 13.6.2 Analyzing Decode-1 with XFLR5: Control Surfaces 221Contents xiii 13.6.3 Analyzing Decode-1 with XFLR5: Stability 223 13.6.4 Flight Simulators 227 13.6.5 Analyzing Decode-1 with Fluent 228 14 Preliminary Structural Analysis 237 14.1 Structural Modeling Using AirCONICS 240 14.2 Structural Analysis Using Simple Beam Theory 243 14.3 Finite Element Analysis (FEA) 245 14.3.1 FEA Model Preparation 246 14.3.2 FEA Complete Spar and Boom Model 250 14.3.3 FEA Analysis of 3D Printed and Fiber- or Mylar-clad Foam Parts 255 14.4 Structural Dynamics and Aeroelasticity 265 14.4.1 Estimating Wing Divergence, Control Reversal, and Flutter Onset Speeds 266 14.5 Summary of Preliminary Structural Analysis 272 15 Weight and Center of Gravity Control 273 15.1 Weight Control 273 15.2 Longitudinal Center of Gravity Control 279 16 Experimental Testing and Validation 281 16.1 Wind Tunnels Tests 282 16.1.1 Mounting the Model 282 16.1.2 Calibrating the Test 284 16.1.3 Blockage Effects 284 16.1.4 Typical Results 287 16.2 Airframe Load Tests 290 16.2.1 Structural Test Instruments 290 16.2.2 Structural Mounting and Loading 293 16.2.3 Static Structural Testing 294 16.2.4 Dynamic Structural Testing 296 16.3 Avionics Testing 300 17 Detail Design: Constructing Explicit Design Geometry 303 17.1 The Generation of Geometry 303 17.2 Fuselage 306 17.3 An Example UAV Assembly 309 17.3.1 Hand Sketches 311 17.3.2 Master Sketches 311 17.4 3D Printed Parts 313 17.4.1 Decode-1: The Development of a Parametric Geometry for the SLS Nylon Wing Spar/Boom “Scaffold Clamp” 313 17.4.2 Approach 314 17.4.3 Inputs 314 17.4.4 Breakdown of Part 315 17.4.5 Parametric Capability 316xiv Contents 17.4.6 More Detailed Model 317 17.4.7 Manufacture 318 17.5 Wings 318 17.5.1 Wing Section Proile 320 17.5.2 Three-dimensional Wing 323 PART IV MANUFACTURE AND FLIGHT 18 Manufacture 331 18.1 Externally Sourced Components 331 18.2 Three-Dimensional Printing 332 18.2.1 Selective Laser Sintering (SLS) 332 18.2.2 Fused Deposition Modeling (FDM) 335 18.2.3 Sealing Components 335 18.3 Hot-wire Foam Cutting 337 18.3.1 Fiber and Mylar Foam Cladding 339 18.4 Laser Cutting 339 18.5 Wiring Looms 342 18.6 Assembly Mechanisms 342 18.6.1 Bayonets and Locking Pins 345 18.6.2 Clamps 346 18.6.3 Conventional Bolts and Screws 346 18.7 Storage and Transport Cases 347 19 Regulatory Approval and Documentation 349 19.1 Aviation Authority Requirements 349 19.2 System Description 351 19.2.1 Airframe 352 19.2.2 Performance 355 19.2.3 Avionics and Ground Control System 356 19.2.4 Acceptance Flight Data 358 19.3 Operations Manual 358 19.3.1 Organization, Team Roles, and Communications 359 19.3.2 Brief Technical Description 359 19.3.3 Operating Limits, Conditions, and Control 359 19.3.4 Operational Area and Flight Plans 360 19.3.5 Operational and Emergency Procedures 360 19.3.6 Maintenance Schedule 360 19.4 Safety Case 361 19.4.1 Risk Assessment Process 362 19.4.2 Failure Modes and Effects 362 19.4.3 Operational Hazards 363 19.4.4 Accident List 364Contents xv 19.4.5 Mitigation List 364 19.4.6 Accident Sequences and Mitigation 366 19.5 Flight Planning Manual 368 20 Test Flights and Maintenance 369 20.1 Test Flight Planning 369 20.1.1 Exploration of Flight Envelope 369 20.1.2 Ranking of Flight Tests by Risk 370 20.1.3 Instrumentation and Recording of Flight Test Data 370 20.1.4 Pre-light Inspection and Checklists 371 20.1.5 Atmospheric Conditions 371 20.1.6 Incident and Crash Contingency Planning, Post Crash Safety, Recording, and Management of Crash Site 371 20.2 Test Flight Examples 375 20.2.1 UAS Performance Flight Test (MANUAL Mode) 375 20.2.2 UAS CoG Flight Test (MANUAL Mode) 377 20.2.3 Fuel Consumption Tests 377 20.2.4 Engine Failure, Idle, and Throttle Change Tests 377 20.2.5 Autonomous Flight Control 378 20.2.6 Auto-Takeoff Test 380 20.2.7 Auto-Landing Test 380 20.2.8 Operational and Safety Flight Scenarios 381 20.3 Maintenance 381 20.3.1 Overall Airframe Maintenance 382 20.3.2 Time and Flight Expired Items 382 20.3.3 Batteries 383 20.3.4 Flight Control Software 383 20.3.5 Maintenance Record Keeping 384 21 Lessons Learned 385 21.1 Things that Have Gone Wrong and Why 388 PART V APPENDICES, BIBLIOGRAPHY, AND INDEX A Generic Aircraft Design Flowchart 395 B Example AirCONICS Code for Decode-1 399 C Worked (Manned Aircraft) Detail Design Example 425 C.1 Stage 1: Concept Sketches 425 C.2 Stage 2: Part Deinition 429 C.3 Stage 3: “Flying Surfaces” 434 C.4 Stage 4: Other Items 435 C.5 Stage 5: Detail Deinition 435 Bibliography 439 Index 441List of Figures Figure 1.1 The University of Southampton UAV team with eight of our aircraft, March 2015. See also https://www.youtube.com/c/SotonUAV and https://www.sotonuav.uk/. 4 Figure 1.2 The design spiral. 7 Figure 2.1 The Southampton University SPOTTER aircraft at the 2016 Farnborough International Airshow. 19 Figure 2.2 University of Southampton SPOTTER UAV with under-slung payload pod. 20 Figure 2.3 Integral fuel tank with trailing edge lap and main spars. 21 Figure 2.4 A typical carbon spar and foam wing with SLS nylon ribs at key locations (note the separate aileron and lap with associated servo linkages). 22 Figure 2.5 A typical SLS structural component. 23 Figure 2.6 A typical integral fuel tank. 25 Figure 2.7 Typical telemetry data recorded by an autopilot. Note occasional loss of contact with the ground station recording the data, which causes the signals to drop to zero. 26 Figure 2.8 Flight tracks of the SPOTTER aircraft while carrying out automated takeoff and landing tests. A total of 23 fully automated lights totaling 55 km of lying is shown. 26 Figure 2.9 A typical UAV wiring diagram. 28 Figure 2.10 The SkyCircuits SC2 autopilot (removed from its case). 29 Figure 2.11 University of Southampton SPOTTER UAV with under-slung maritime light releasable AUV. 29 Figure 3.1 Variation of airfoil section drag at zero lift with section Reynolds number and thickness-to-chord ratio. After Hoerner [9]. 35 Figure 3.2 A UAV with signiicant FDM ABS winglets (this aircraft also has Custer ducted fans). 36 Figure 3.3 Wing foam core prior to covering or rib insertion –note strengthened section in way of main wing spar. 36xviii List of Figures Figure 3.4 Covered wing with spar and rib – in this case, the rib just acts to transfer the wing twisting moment while the spar is bonded directly to the foam without additional strengthening. 37 Figure 3.5 A SPOTTER UAV wing spar under static sandbag test. 38 Figure 3.6 SLS nylon wing rib with spar hole – note the extended load transfer elements that are bonded to the main foam parts of the wing and also lap hinge point. 39 Figure 3.7 Two wing foam cores with end rib and spar inserted – note in this case the rib does not extend to the rear of the section, as a separate wing morphing mechanism will be itted to the rear of the wing. 39 Figure 3.8 SPOTTER UAV wing under construction showing the two-part aileron plus lap, all hinged off a common rear wing spar – note also the nylon torque peg on the rib nearest the camera. 40 Figure 3.9 UAV that uses wing warping for roll control. 41 Figure 3.10 UAV that uses tiperons for roll control. 41 Figure 3.11 Fowler lap – note the complex mechanism required to deploy the lap. 42 Figure 3.12 Simple FDM-printed wing tip incorporated into the outermost wing rib. 43 Figure 3.13 UAV with pneumatically retractable undercarriage – the main wheels retract into the wings while the nose wheel tucks up under the fuselage (wing cut-out shown prior to undercarriage installation). 43 Figure 3.14 Integral fuel tank in central wing section for SPOTTER UAV. 44 Figure 4.1 SPOTTER SLS nylon engine nacelle/fuselage and interior structure. 46 Figure 4.2 Bayonet system for access to internal avionics (a) and fuselage-mounted switch and voltage indicators (b). 46 Figure 4.3 Load spreader plate on Mylar-clad foam core aileron. 46 Figure 4.4 Commercially produced model aircraft with foam fuselage (and wings). 47 Figure 4.5 Space frame structure made of CFRP tubes with SLS nylon joints and foam cladding. 47 Figure 4.6 DECODE aircraft with modular fuselage elements. 48 Figure 4.7 Wing attachment on SPOTTER fuselage. Note the recess for square torque peg with locking pin between main and rear spar holes. 48 Figure 4.8 Typical engine and motor mounts for SLS nylon fuselages and nacelles. Note the steel engine bearer in irst view, engine hours meter in second image, and vibration isolation in third setup. 49 Figure 4.9 Frustratingly small fuselage access hatch. 50 Figure 4.10 Typical plywood avionics boards with equipment mounted. Note dual layer system with antivibration mounts in last image. 50 Figure 4.11 SULSA forward-looking video camera. 51 Figure 4.12 SPOTTER payload pods with ixed aperture for video camera (a) and downward and sideways cameras (b and c). 51List of Figures xix Figure 4.13 Simple two axis gimbal system and Hero2 video camera mounted in front of nose wheel. 52 Figure 4.14 Three-axis gimbal system and Sony video camera mounted in front of the nose wheel. Note the video receiver system on the bench that links to the camera via a dedicated radio channel. 53 Figure 4.15 SPOTTER integral fuel tank. Note internal bafle and very small breather port (top left) in the close-up view of the iller neck. 53 Figure 4.16 Aircraft with SLS nylon fuselage formed in three parts: front camera section attached by bayonet to rear two sections joined by tension rods. Note the steel tension rod inside the hull just behind bayonet in the right-hand image. 54 Figure 4.17 Example hatches in SLS nylon fuselages. Note the locking pins and location tabs on the right-hand hatch. 55 Figure 4.18 Metal-reinforced nose wheel attachment with steering and retract hinge in aluminum frame attached to SLS nylon fuselage. Note the nose wheel leg sized to protect the antenna. 56 Figure 4.19 Nylon nose wheel attachment. Note the signiicant reinforcement around the lower and upper strut bearings. 56 Figure 4.20 Tails attached directly to the fuselage. The right-hand aircraft is a heavily modiied commercial kit used for piggy-back launches of gliders. 57 Figure 4.21 Tails attached using CFRP booms, both circular and square in cross-section. 57 Figure 4.22 All-moving horizontal stabilizer with port/starboard split to augment roll control and provide redundancy. 57 Figure 4.23 SLS nylon part to attach tail surfaces to a CFRP tail boom. 58 Figure 5.1 UAV engine/electric motor/propeller test cell. Note the starter generator on the engine behind the four-bladed propeller. 60 Figure 5.2 UAV engine dynamometer. 61 Figure 5.3 Typical maximum powers, weights, and estimated peak static thrusts of engines for UAVs in the 2–150 kg MTOW range. 61 Figure 5.4 OS Gemini FT-160 glow-plug engine in pusher coniguration. Note the permanent wiring for glow-plugs. 62 Figure 5.5 OS 30 cc GF30 four-stroke engine installed in a hybrid powered UAV. Note the signiicant size of the exhaust system. 63 Figure 5.6 Saito 57 cc twin four-stroke engine in pusher coniguration. Note the pancake starter generator itted to this engine. 64 Figure 5.7 Twin 3W-28i CS single-cylinder two-stroke engines itted to 2Seas UAV. Note again the signiicant size of the exhaust systems. 64 Figure 5.8 Twin OS 40 cc GF40 four-stroke engines installed in SPOTTER UAV, with and without engine cowlings. 64 Figure 5.9 Raw performance data taken from an engine under test in our dynamometer. 66xx List of Figures Figure 5.10 Hacker brushless electric motor. 67 Figure 5.11 Outputs from JavaProp “multi analysis” for a propeller operating at ixed torque. Note the differing horizontal scales. Note the design point: here a typical cruise speed of 25 m/s is shown by the small circle and is slightly below the peak eficiency for the design. Peak thrust occurs at about 4 m/s, ensuring a good ability to start the aircraft rolling on a grass ield. 69 Figure 5.12 Large UAV fuel tanks. Note clunks and fuel level sensor itting at rear left-hand corner of one tank. 70 Figure 5.13 SPOTTER fuel tank level sensors. One sensor lies behind the central lap in the upper wider part of the tank (just visible in the right-hand image), while the second one lies at the bottom just above the payload interface. 71 Figure 5.14 Engine-powered brushless generators driven directly or by toothed belt. 72 Figure 6.1 Outline avionics diagram for SPOTTER UAV. 74 Figure 6.2 Outline avionics diagram for SPOTTER UAV (detail) – note switch-over unit linking dual receivers and dual autopilots. 75 Figure 6.3 Typical avionics boards. Note the use of MilSpec connectors (the Futaba receivers are marked 1, the switch-over unit 2, the SC2 autopilot and GPS antenna 3, and the avionics and ignition batteries 4 and 5, respectively). 76 Figure 6.4 Fuselage with externally visible LED voltage monitor strips. Here, one is for the avionics system and the second for the ignition system. 77 Figure 6.5 Aircraft with twin on-board, belt-driven generators as supplied by the UAV Factory and a close-up of UAV Factory system. 77 Figure 6.6 On-board, belt-driven brushless motor used as generators. 78 Figure 6.7 Aircraft with a Sullivan pancake starter–generator system. 78 Figure 6.8 A selection of aircraft servos from three different manufacturers. 79 Figure 6.9 Variation of servo torque with weight for various manuafcturers’ servos. 80 Figure 6.10 SPOTTER aircraft showing multiple redundant ailerons and elevators. 81 Figure 6.11 Servo cut-out in wing with SLS nylon reinforcement box. 81 Figure 6.12 Typical servo linkage. Note the servo arm, linkage, and servo horn (with reinforcing pad). 82 Figure 6.13 SPOTTER “iron bird” test harness layout. Note the full-size airframe drawing placed under the wiring. 83 Figure 6.14 Generator and drive motor for “iron bird” testing. 83 Figure 6.15 SPOTTER “iron bird” with resulting professionally built harness in place. 84 Figure 6.16 Decode-1 “iron bird” with harness that uses simple aero-modeler-based cable connections. 85 Figure 6.17 Baseboard with mil spec connections on left- and right-hand edges. Note SkyCircuits SC2 autopilot itted top right with GPS antenna on top and switch-over unit in the center with very many wiring connections. 85 Figure 6.18 Laser-cut plywood baseboards. 86List of Figures xxi Figure 6.19 Components located directly into 3D SLS nylon printed structure. The servo is screwed to a clip-in SLS part, while the motor is bolted directly to the fuselage. 86 Figure 6.20 Basic Arduino Uno autopilot components including GPS module on extension board, and accelerometer, barometer and three-axis gyro on daughter boards. 87 Figure 6.21 Pixhawk autopilot. 87 Figure 6.22 The SkyCircuits SC2 autopilot (removed from its case (a), and with attached aerials and servo connection daughter board (b). See also Figure 6.3, where the SC2 is itted with its case and a GPS aerial on top). 88 Figure 6.23 A selection of professional-grade 5.8 GHz video radiolink equipment: (front) transmitter with omnidirectional antenna in ruggedized case, and (rear left to right) receiver, directional antenna, and combined receiver/high intensity screen unit. 89 Figure 6.24 A hobby-grade 5.8 GHz video radiolink: (a) receiver with omnidirectional antenna and (b) transmitter with similar unclad antenna and attached mini-camera. 89 Figure 6.25 Futaba s-bus telemetry modules:(clockwise from left) temperature sensor, rpm sensor, and GPS receiver. 90 Figure 7.1 Some typical small UAV undercarriages. 94 Figure 7.2 An aircraft with spats itted to its main wheels to reduce drag. 94 Figure 7.3 Nose wheel and strut showing suspension elements, main bearings, control servo, and caster. 95 Figure 7.4 Tail wheel showing suspension spring. 96 Figure 7.5 Nose wheel mechanism with combined spring-coupled steering and vertical suspension spring. 96 Figure 7.6 UAV with a pneumatic, fully retractable undercarriage system. Note also the nose camera that has been added to the aircraft shown in the image with undercarriage retracted. 97 Figure 7.7 Details of fully retractable undercarriage system. 97 Figure 8.1 Explosion of information content as design progresses. 102 Figure 8.2 2SEAS aircraft with redundant ailerons and elevators. 109 Figure 8.3 Autopilot system on vibration test. 109 Figure 8.4 Treble isolated engine mounting. 110 Figure 8.5 Typical military UAV work-breakdown structure interface deinitions, from MIL-HDBK-881C for UAVs. 111 Figure 8.6 Example military system requirements lowdown [13]. Defence Acquisition University. 114 Figure 8.7 Systems engineering “V” model. 114 Figure 8.8 Weight prediction of SPOTTER UAV. 115xxii List of Figures Figure 8.9 Pie chart plots of SPOTTER weight. 116 Figure 8.10 Example weight and cost breakdown. 117 Figure 9.1 Outline design worklow. 121 Figure 9.2 Analysis tool logic. 122 Figure 9.3 Mission analysis using the AnyLogic event-driven simulation environment. 126 Figure 10.1 On May 14, 1954, Boeing oficially rolled out the Dash-80, the prototype of the company’s 707 jet transport. Source: This photo, by John M. ‘Hack’ Miller, was taken during the rollout (Image courtesy of the Museum of History & Industry, Seattle https://creativecommons. org/licenses/by-sa/2.0/ – no copyright is asserted by the inclusion of this image). 131 Figure 10.2 Four semi-randomly chosen points in an immense space of unmanned aircraft topologies: (starting at the top) the Scaled Composites Proteus, the NASA Prandtl-D research aircraft, the AeroVironment RQ-11 Raven, and the NASA Helios (images courtesy of NASA and the USAF). 134 Figure 10.3 Minimum mass cantilever designed to carry a point load. 135 Figure 10.4 NASA oblique-wing research aircraft (images courtesy of NASA). Could your design beneit from asymmetry? 138 Figure 10.5 Multifunctionality: the 3D-printed fuel tank (highlighted) of the SPOTTER unmanned aircraft does not only hold the fuel (with integral bafles) but also generates lift and it has a structural role too, see also Figures 2.3, 2.6, and 3.14. 140 Figure 10.6 Typical constraint diagram. Each constraint “bites” a chunk out of the P versus W∕S space; whatever is left is the feasible region, wherein the design will have to be positioned. 163 Figure 11.1 Decode-1 in the R.J. Mitchell wind tunnel with wheels and wing tips removed and electric motor for propeller drive. 175 Figure 11.2 Decode-1 in light with nose camera itted. 175 Figure 11.3 Decode-1 spreadsheet snapshot – inputs page. 178 Figure 11.4 Decode-1 spreadsheet snapshot – results summary page. 179 Figure 11.5 Decode-1 spreadsheet snapshot – geometry page. 180 Figure 11.6 Decode-1 outer geometry as generated with the AirCONICS tool suite. 180 Figure 11.7 Decode-2 spreadsheet snapshot. 183 Figure 11.8 Decode-2 in light with nose camera itted. 183 Figure 11.9 Decode-2 outer geometry as generated with the AirCONICS tool suite. 184 Figure 11.10 SPOTTER spreadsheet snapshot. 186 Figure 11.11 SPOTTER in light with payload pod itted. 187 Figure 11.12 SPOTTER outer geometry as generated with the AirCONICS tool suite. 187 Figure 12.1 Basic AirCONICS airframe geometry for a single tractor engine, twin-boom, H-tail design. 191List of Figures xxiii Figure 12.2 AirCONICS model of complete Decode-1 airframe with control surfaces, undercarriage, and propeller disk. 192 Figure 13.1 Cp and streamline plot for the NACA0012 foil at 16∘ angle of attack as computed with XFoil. 197 Figure 13.2 Results of XFoil analysis sweep for the NACA 64–201 foil at Mach 0.17 as computed with XFLR5. 199 Figure 13.3 Results of XFLR5 analysis sweep for a wing generated from the NACA 64–201 foil sections at Reynold’s number of 4.4 million and Mach 0.17. 201 Figure 13.4 Convergence plot of two-dimensional k-� SST RANS-based CFD analysis. 203 Figure 13.5 Pathlines and surface static pressure plot from Fluent RANS based CFD solution. 203 Figure 13.6 Section through a coarse-grained 3D Harpoon mesh for typical Spalart–Allmaras UAV wing model and close-up showing a boundary layer mesh. 206 Figure 13.7 Histogram of y+ parameter for typical boundary layer mesh using the Spalart–Allmaras one-parameter turbulence model. 207 Figure 13.8 Histogram of y+ parameter for typical boundary layer mesh using the k − � SST turbulence model. 207 Figure 13.9 Lift versus drag polar for NAC0012 airfoil from XFoil and experiments. Note that when plotted in this way, both lift and drag coeficients may be found at a given angle of attack or, for a given lift coeficient, drag coeficient and angle of attack may easily be read off. 208 Figure 13.10 Low-resolution NASA Langley 2D mesh around the NACA0012 foil. 209 Figure 13.11 Middle-resolution NASA Langley 2D mesh around the NACA0012 foil. 209 Figure 13.12 ICEM 2D mesh around the NACA0012 foil (courtesy of Dr D.J.J. Toal). 210 Figure 13.13 Experimental and 2D computational lift and drag data for the NACA0012 airfoil (using the k − � SST turbulence model). Adapted from Abbott and von Doenhoff 1959. 210 Figure 13.14 Computed two-dimensional low past the NACA0012 foil when almost fully stalled. 211 Figure 13.15 Section through a ine-grained Harpoon 3D mesh around the NACA0012 foil suitable for the k − � SST turbulence model. Note the wake mesh extending from the trailing edge. 211 Figure 13.16 Experimental and 3D computational lift and drag data for the NACA0012 airfoil (using the Spalart–Allmaras and k − � SST turbulence models). NASA. 212 Figure 13.17 Experimental [20, 22] and computational lift and drag data for the NACA 64–210 section. 213 Figure 13.18 Pathlines from a RANS k − � solution for the NACA 64–210 airfoil at 12∘ angle of attack. Note the reversed low and large separation bubble on the upper surface. 214xxiv List of Figures Figure 13.19 Experimental and computational lift and drag data for the Sivells and Spooner [21] wing and y+ for the k − � SST Harpoon mesh. NASA. 215 Figure 13.20 XFLR5 model of the Sivells and Spooner wing. 216 Figure 13.21 Experimental and computational lift and drag data for the Sivells and Spooner [21] wing with enhanced k − � SST Harpoon mesh of 76 million cells and y+ for the enhanced mesh. 217 Figure 13.22 Pathlines and static pressure around the Sivells and Spooner [21] wing with enhanced k − � SST Harpoon mesh at 11∘ angle of attack. NASA. 218 Figure 13.23 XFLR5 model of Decode-1 airframe as generated by AirCONICS with main wing setting angle of 0∘ and elevator setting angle of −2.85∘, at an angle of attack of 2.6∘ and 30 m/s. Note the use of cambered sections for the main wing and symmetrical proiles for the elevator and ins. The green bars indicate the section lift, with the tail producing downforce to ensure pitch stability. 218 Figure 13.24 XFLR5-generated polar plot for Decode-1 airframe as generated by AirCONICS with main wing setting angle of 0∘ and elevator setting angle of −2.85∘, showing speed variations from 15 to 30 m/s. The black circles indicate light at an angle of attack of 2.53∘ at which Cm is zero. 219 Figure 13.25 XFLR5-generated polar plot for Decode-1 airframe as generated by AirCONICS with main wing setting angle of 2.53∘ and elevator setting angle of −0.34∘, showing speed variations from 15 to 30 m/s. Note that Cl is 0.28 and Cm is zero at an angle of attack of 0∘ as required in the cruise condition. 220 Figure 13.26 XFLR5-generated polar plot for Decode-1 airframe at 30 m/s with main wing setting angle of 0∘, showing variations in center of gravity position by 100 mm, reduction in tail length by 300 mm, and elevator set at an angle of 0∘. 222 Figure 13.27 Time-domain simulation for XFLR5-generated eigenvalues at 30 m/s taken from Table 13.2 showing �R for the roll mode and T2 for the spiral mode. 228 Figure 13.28 University of Southampton light simulator. 229 Figure 13.29 Decode-1 mesh shown inside Harpoon along with wake surfaces and reinement zones. 230 Figure 13.30 Fluent mesh on the center plane for the Decode-1 airframe k − � SST analysis at 30 m/s, together with resulting y+ histogram. 231 Figure 13.31 Fluent convergence plot for Decode-1 whole aircraft model at 30 m/s. 232 Figure 13.32 Polar plot for Decode-1 airframe at 30 m/s showing both Fluent and XLFR5 results for lift and drag. Those for Fluent include results for just the lifting surfaces and with the complete airframe fuselage, control surfaces, and undercarriage gear; those for XFLR5 show also the impact of adding a ixed parasitic drag coeficient of 0.0375. 232 Figure 13.33 AirCONICS model of Decode-1 lifting surfaces. 233List of Figures xxv Figure 13.34 AirCONICS model of complete Decode-1 airframe with control surfaces, undercarriage, and propeller disk. 233 Figure 13.35 Streamlines colored by velocity magnitude around the complete Decode-1 airframe with delected ailerons. 234 Figure 14.1 Typical composite Vn diagram for gust and maneuver loads on a small UAV (here for Decode-1 assuming maneuver load factors of +5 and −2, 9.1 m/s gust velocity, and a dive speed of 160% of the cruise speed). 238 Figure 14.2 Breakdown of Decode-1 outer mold line model into individual components for structural modeling. 240 Figure 14.3 Decode-1 components that will be produced by 3D printing or made from laser-cut ply. 242 Figure 14.4 Delection and slope variations for the Decode-1 main spar when lying at 30 m/s and an angle of attack of 2.53∘ using loading taken from XFLR5, a load factor of 4, and simple beam theory analysis. The spar is assumed to be made from a circular CFRP section of outer diameter 20 mm, wall thickness 2 mm, Young’s modulus of 70 GPa, and extending the full span of the aircraft, being clamped on the center plane. 245 Figure 14.5 Preliminary spar layout for Decode-1. Here the linking parts are taken directly from AirCONICS without being reduced to either thick-walled or thin-walled rib-reinforced structures. 246 Figure 14.6 Simpliied Abaqus main spar model with solid SLS nylon supports for Decode-1, showing subdivided spar and boundary conditions for a 4g maneuver loading. 247 Figure 14.7 Deformed shape and von Mises stress plot for Decode-1 main spar under 4g light loads using a uniform spar load. The tip delection is 189.7 mm. 248 Figure 14.8 Abaqus loading for full Decode-1 spar model under wing light loads taken from XFLR5 together with a load factor of 4 plus elevator and in loading based on Cl values of unity. 251 Figure 14.9 Deformed shape and von Mises stress plot for full Decode-1 spar model under wing light loads taken from XFLR5 together with a load factor of 4 plus elevator and in loading based on Cl values of unity. The main spar tip delections are 143.9 mm, the elevator spar tip delections are 10.8 mm, and the in spar tip delections are 11.1 mm. 252 Figure 14.10 Further details of the deformed shape and von Mises stress plot for full Decode-1 spar model under wing light loads taken from XFLR5 together with a load factor of 4 plus elevator and in loading based on Cl values of unity. 253 Figure 14.11 Deformed shape and von Mises stress plot for nylon support part in full Decode-1 spar model under wing light loads taken from XFLR5 together with a load factor of 4 plus elevator and in loading based on Cl values of unity. 255xxvi List of Figures Figure 14.12 Deformed shape and von Mises stress plot for full Decode-1 spar model with locally reined mesh under wing light loads taken from XFLR5 together with a load factor of 4 plus elevator and in loading based on Cl values of unity. 256 Figure 14.13 Deformed shape and von Mises stress plot for full Decode-1 spar model with fully reined mesh and reduced boundary conditions under wing light loads taken from XFLR5 together with a load factor of 4 plus elevator and in loading based on Cl values of unity. 257 Figure 14.14 Simpliied Abaqus thick-walled structural model for Decode-1 SLS nylon part. The mesh for this part contains 25 000 elements. 257 Figure 14.15 Deformed shape and von Mises stress plot for thick-walled nylon part in full Decode-1 spar model with fully reined mesh and reduced boundary conditions under wing light loads taken from XFLR5 together with a load factor of 4 plus elevator and in loading based on Cl values of unity. 258 Figure 14.16 Deformed shape and von Mises stress plot for 2 mm thick-walled nylon part in full Decode-1 spar model with fully reined mesh and reduced boundary conditions under wing light loads taken from XFLR5 together with a load factor of 4 plus elevator and in loading based on Cl values of unity. 259 Figure 14.17 Abaqus model of foam core created with CAD shell and illet commands and meshed with brick hex elements. 260 Figure 14.18 Abaqus model of glass-iber wing cover created with CAD shell commands and meshed with continuum shell hex elements. Note the wedge elements used for the sharp trailing edge. 260 Figure 14.19 Abaqus assembly with foam parts added, highlighting the tie constraint between the foam and the SLS nylon support. 261 Figure 14.20 Pressure map on Decode-1 foam part under wing light loads taken from XFLR5. 261 Figure 14.21 Resulting delections and stresses in foam core and cover for wing under light conditions. 263 Figure 14.22 Resulting delections and stresses in SLS nylon part with foam mounting lug for wing under light conditions. 264 Figure 14.23 Two-degrees-of-freedom model of wing aeroelasticity. 267 Figure 14.24 Truncated Abaqus contour plot of a irst twist mode revealing the nodal line and hence the elastic axis. 270 Figure 14.25 Abaqus plots of irst lap and twist modes for Decode-1 wing. 271 Figure 15.1 Channel wing aircraft being weighed after inal assembly. 278 Figure 16.1 Decode-1 and channel wings on wind tunnel mounting rig. Note the circular boundary plate that stands in for the absent fuselage. 283 Figure 16.2 AirCONICS model of Decode-1 airframe in a representation of the R.J. Mitchell 11’ × 8’ wind tunnel working section at Southampton University, illustrating degree of blockage. 285List of Figures xxvii Figure 16.3 AirCONICS half-model of Decode-1 airframe in the R.J. Mitchell 11’ × 8’ wind tunnel prior to mesh preparation. 286 Figure 16.4 Section through Fluent velocity magnitude results and Harpoon mesh for Decode-1 airframe in the R.J. Mitchell 11’ × 8’ wind tunnel. Note the extent of the boundary layer on the tunnel walls and the ine boundary layer mesh needed to resolve this, along with the reinement zone near the wing tip. 286 Figure 16.5 Decode-1 baseline wind tunnel results (control surfaces in neutral positions) under varying wind speed. (a) Lift coeficient. (b) Drag coeficient. (c) Side coeficient. (d) Pitch coeficient. (e) Roll coeficient. (f) Yaw coeficient. 288 Figure 16.6 Decode-1 elevator effectiveness with varying delection angles and wind speed. (a) Lift coeficient at 15 m/s. (b) Drag coeficient at 15 m/s. (c) Pitch coeficient at 10 m/s. (d) Pitch coeficient at 15 m/s. (e) Pitch coeficient at 24 m/s. 289 Figure 16.7 Decode-1 rudder effectiveness with varying delection angle. (a) Lift coeficient at 24 m/s. (b) Drag coeficient at 24 m/s. (c) Side coeficient at 24 m/s. (d) Pitch coeficient at 24 m/s. (e) Roll coeficient at 24 m/s. (f) Yaw coeficient at 24 m/s. 291 Figure 16.8 Dial gauge in use to measure aiframe delection during static test in the lab. 292 Figure 16.9 Lab-quality force transducer, piezoelectric accelerometers, and electromagnetic shakers. 293 Figure 16.10 Flight-capable piezoelectric accelerometer and data-capture system. 293 Figure 16.11 Mounting system for wing and main spar assembly under sandbag load test. 294 Figure 16.12 Clamping system for main spar. 294 Figure 16.13 Wing assembly under sandbag load test. 295 Figure 16.14 Partial failure of SLS nylon structural component during sandbag load test. Note the signiicant cracks and large deformations. 296 Figure 16.15 Load testing of an undercarriage leg and associated SLS nylon mounting structure. Note the dummy carbon-iber tubes present to allow the SLS structure to be correctly set up. 296 Figure 16.16 Ground vibration test of a Decode-1 wing showing support and mounting arrangements. 297 Figure 16.17 Ground vibration test of a Decode-1 wing ((a) accelerometer on starboard wing tip: (b) shaker and force transducer near wing root). 298 Figure 16.18 Frequency response from ground vibration test of a Decode-1 wing: accelerometer on port wing tip and cursors on irst lap mode. 298 Figure 16.19 Frequency response from ground vibration test of a Decode-1 wing: lapping mode accelerometer placement (upper) and twisting mode placement (lower), cursors on irst twist mode. 299xxviii List of Figures Figure 16.20 SPOTTER iron-bird being used to test a complete avionics build-up: note motors to spin generators in a realistic manner. 301 Figure 16.21 Avionics board under vibration test. Note the free-free mounting simulated by elastic band supports. In this case, a force transducer has been placed between the shaker and the long connecting rod that stimulates the board. The in-built accelerometer in the light controller is used to register motions. 301 Figure 16.22 Typical Servo test equipment: (front left to right) simple low-cost tester, large servo, motor speed tester with in-built power meter, and servo control output; (rear) avionics battery and standard primary receiver. 302 Figure 17.1 Detail design process low. 304 Figure 17.2 The structure of well-partitioned concept design models. 305 Figure 17.3 Example coniguration studies. 306 Figure 17.4 Example 3D models of Rotax aircraft engine and RCV UAV engine. Courtesy of Chris Bill and RCV Engines Ltd. 307 Figure 17.5 Example of images used to create realistic looking 3D Solidworks geometry model. 308 Figure 17.6 2D side elevations of Rotax aircraft engine and RCV UAV engine. Courtesy of Chris Bill and RCV Engines Ltd. 308 Figure 17.7 Scaling dimension added to drawing (mm). Courtesy of Chris Bill. 308 Figure 17.8 “Spaceframe” aircraft structure. 309 Figure 17.9 Illustrative student UAV assembly. 310 Figure 17.10 UAV assembly model can be modiied by changing design table parameters. 310 Figure 17.11 Plan and side view hand sketches. 311 Figure 17.12 Hand sketch scaled and positioned orthogonally in Solidworks. 312 Figure 17.13 Exact, dimensioned sketch being created on hand-sketch outline. 312 Figure 17.14 The “master” driving sketches in the assembly. 313 Figure 17.15 Design table for example UAV. 313 Figure 17.16 Input reference geometry. 314 Figure 17.17 The input geometry modeled as partitioned parts. 315 Figure 17.18 The assembly generated from reference geometry. 315 Figure 17.19 “Debugging” the detailed model. 316 Figure 17.20 Trimming of boom tube fairing. 317 Figure 17.21 Final detailed model. 318 Figure 17.22 Multipanel wing of PA-28. Photo courtesy Bob Adams https://creativecommons.org/licenses/by-sa/2.0/ – no copyright is asserted by the inclusion of this image. 319 Figure 17.23 NACA four-digit section coordinate spreadsheet. 320 Figure 17.24 Curve importing in Solidworks. 321List of Figures xxix Figure 17.25 Use of “convert entities” in Solidworks. 321 Figure 17.26 Closing the 2D aerofoil shape. 322 Figure 17.27 Deleting sketch relationship with reference geometry. 322 Figure 17.28 Reference geometry. 323 Figure 17.29 Constraining curve to reference “scaffold” geometry. 323 Figure 17.30 “3D” scaffold to deine the relative positions in space of two independently scalable wing sections. 324 Figure 17.31 Wing surface with span, twist, taper, and sweep variables. 324 Figure 17.32 Multipanel wing. 325 Figure 17.33 Example of a double-curvature composite wing. 325 Figure 17.34 Fabricated wing structures. 326 Figure 17.35 Simple wooden rib and alloy spar structure. 326 Figure 17.36 Parametric wing structure. 327 Figure 18.1 3D SLS nylon parts as supplied from the manufacturer. 332 Figure 18.2 3D SLS stainless steel gasoline engine bearer after printing and in situ. 333 Figure 18.3 3D SLS nylon manufacturing and depowdering. 333 Figure 18.4 Small ofice-based FDM printer. Parts as they appear on the platten and after removal of support material. 335 Figure 18.5 FDM-printed ABS fuselage parts. 336 Figure 18.6 Aircraft with FDM-printed fuselage and wing tips. 336 Figure 18.7 In-house manufactured hot-wire foam cutting machine. This cuts blocks of foam up to 1400 mm × 590 mm × 320 mm. 338 Figure 18.8 Large hot-wire foam cutting machine. 338 Figure 18.9 Hot-wire-cut foam wing parts: (Left) The original material blocks with and without cores removed; (right) with FDM-manufactured ABS joining parts. 339 Figure 18.10 Foam wings after cladding: glass iber, Mylar, and illed glass iber. 340 Figure 18.11 Aircraft with wings fabricated from laser-cut plywood covered with aero-modeler ilm. 341 Figure 18.12 Avionics base board and servo horn reinforcement made from laser cut plywood. 341 Figure 18.13 Foam reinforcement ribs made from laser-cut plywood. 341 Figure 18.14 Logical wiring diagram (detail). 343 Figure 18.15 Iron bird for building wiring looms. 344 Figure 18.16 Soldering station (note the clamps, heat-resistant mat, and good illumination). 344 Figure 18.17 Female and male bayonet produced in SLS nylon with quick-release locking pin. 345xxx List of Figures Figure 18.18 Quick-release pin itting used to retain a wing to a fuselage (note lug on wing rib). 345 Figure 18.19 SLS nylon clamping mechanisms. 346 Figure 18.20 Cap-screws and embedded retained nuts, here on an undercarriage ixing point. 347 Figure 18.21 Transport and storage cases. 347 Figure 19.1 Typical take-off performance. 356 Figure 19.2 Typical wiring schematic. 357 Figure 20.1 Typical light log. 370 Figure 20.2 Typical pre-light checklist. 372 Figure 20.3 Typical light procedures checklist. 373 Figure 21.1 Our irst student-designed UAV. 386 Figure 21.2 Not all test lights end successfully! 386 Figure 21.3 Aircraft with variable length fuselage. (a) Fuselage split open. (b) Spare fuselage section. 387 Figure 21.4 Student-designed lying boat with large hull volume forward and insuficient vertical tail volume aft. 389 Figure 21.5 Aircraft with split all-moving elevator. (a) Without dividing fence. (b) With fence. 389 Figure 21.6 Autopilot on vibration test. 390 Figure 21.7 Student UAV with undersized wings. The open payload bay also added to stability issues. 390 Figure 21.8 2SEAS aircraft after failure of main wheel axle. 391 Figure A.1 Generic aircraft design lowchart. 396 Figure C.1 Vans RV7 Aircraft. Cropped image courtesy Daniel Betts https:// creativecommons.org/licenses/by-sa/2.0/ – no copyright is asserted by the inclusion of this image. 426 Figure C.2 Concept sketches of an aircraft. 426 Figure C.3 Side elevation hand sketch imported and scaled. 426 Figure C.4 Plan, side, and front view imported and scaled. 427 Figure C.5 Tracing the outline of the hand sketch to capture the “essential” geometry. 427 Figure C.6 Dimensioned parametric geometry sketch. 428 Figure C.7 View of all three of the dimensioned parametric geometry sketches. 428 Figure C.8 Center fuselage part, with side elevation parametric geometry sketch in the background. 429 Figure C.9 Underlying geometry for the center fuselage. 429 Figure C.10 Completion of center fuselage. 430 Figure C.11 Rear fuselage synchronized with center fuselage at shared interface. 430 Figure C.12 Fully realized fuselage geometry. 431List of Figures xxxi Figure C.13 Two-panel wing and wing incidence and location line in side elevation. 431 Figure C.14 All the major airframe surface parts added. 431 Figure C.15 Checking against original sketch. 432 Figure C.16 Addition of propeller disk and spinner so that ground clearance can be checked. 432 Figure C.17 Engine installation checking cowling clearance and cooling (note: lightweight decal engine geometry). 433 Figure C.18 Checking the instrument panel it (again use of decal for instruments). 433 Figure C.19 Checking the ergonomics of crew seating and canopy clearance/view. 433 Figure C.20 Hand sketches–to parameterized sketches–to solid assembly. 434 Figure C.21 Whole aircraft parametric variables. 436 Figure C.22 Wing geometry used to calculate lift centers for static margin calculations. 437 Figure C.23 Final parametric aircraft design with all major masses added. 437 Figure C.24 Final detailed geometry. Courtesy of Vans Aircraft, Inc. 438List of Tables Table 1.1 Design system maturity. 9 Table 2.1 Different levels of UAV autonomy classiied using the Wright–Patterson air force base scheme. 16 Table 5.1 Typical liquid-fueled IC engine test recording table (maximum rpms are of course engine-dependent). 66 Table 5.2 Typical IC engine BMEP values taken from various sources. 67 Table 6.1 Typical primary transmitter/receiver channel assignments. 76 Table 6.2 Typical servo properties. 79 Table 8.1 Example responsibility allocation matrix for a maintenance team. 113 Table 9.1 Concept design requirements. 124 Table 11.1 Typical ixed parameters in concept design. 167 Table 11.2 Typical limits on variables in concept design. 168 Table 11.3 Estimated secondary airframe dimensions. 168 Table 11.4 Variables that might be used to estimate UAV weights. 171 Table 11.5 Items for which weight estimates may be required and possible dependencies. 172 Table 11.6 Other items for which weight estimates may be required. 173 Table 11.7 Design brief for Decode-1. 176 Table 11.8 Resulting concept design from spreadsheet analysis for Decode-1. 176 Table 11.9 Design geometry from spreadsheet analysis for Decode-1 (in units of mm and to be read in conjunction with Tables 11.3 and 11.8). 177 Table 11.10 Design brief for Decode-2. 181 Table 11.11 Estimated secondary airframe dimensions for Decode-2. 181 Table 11.12 Resulting concept design from spreadsheet analysis for Decode-2. 181 Table 11.13 Design geometry from spreadsheet analysis for Decode-2 (in units of mm and to be read in conjunction with Tables 11.11 and 11.12). 184 Table 11.14 Design brief for SPOTTER. 184 Table 11.15 Estimated secondary airframe dimensions for SPOTTER. 185xxxiv List of Tables Table 11.16 Resulting concept design from spreadsheet analysis for SPOTTER. 185 Table 11.17 Design geometry from spreadsheet analysis for SPOTTER (in units of mm and to be read in conjunction with Tables 11.15 and 11.16). 186 Table 13.1 A summary of some of the Fluent turbulence models based on information provided in ANSYS training materials. 202 Table 13.2 Decode-1 eigenvalues as calculated from XFLR5 stability derivatives using the formulae provided by Phillips [24, 25] and the estimated inertia properties for a light speed of 30 m/s and MTOW of 15 kg. 227 Table 14.1 Shear forces (Q), bending moments (M), slopes (�, in radians), and delections (�) for Euler–Bernoulli analysis of uniform encastre cantilever beams. 243 Table 14.2 A selection of results from various Abaqus models of the Decode-1 airframe. 254 Table 14.3 Natural frequency results (Hz) using Abaqus modal analysis for the Decode-1 airframe. 271 Table 15.1 Typical weight and LCoG control table (LCoG is mm forward of the main spar). 274 Table 18.1 Typical properties of carbon-iber-reinforced plastic (CFRP) tubes. 332 Table 18.2 Typical properties of SLS nylon 12. 334 Table 18.3 Typical properties of closed-cell polyurethane loor insulation foam. 337 Table 18.4 Typical properties of glass-iber-reinforced plastics. 340 Table 19.1 Typical small UAS operations manual template part Ai. 351 Table 19.2 Typical small UAS operations manual template part Aii. 352 Table 19.3 Typical small UAS operations manual template part Bi. 353 Table 19.4 Typical small UAS operations manual template parts Bii, C, and D. 354 Table 19.5 Typical summary airframe description. 355 Table 19.6 Typical engine characteristics. 355 Table 19.7 Typical aircraft performance summary in still air. 356 Table 19.8 Radio control channel assignments. 358 Table 19.9 Risk probability deinitions (igures refer to light hours). 361 Table 19.10 Accident severity deinitions. 361 Table 19.11 Risk classiication matrix. 362 Table 19.12 Risk class deinitions. 362 Table 19.13 Typical failure effects list (partial). 363 Table 19.14 Typical hazard list (partial). 364 Table 19.15 Typical accident list (partial). 365 Table 19.16 Typical mitigation list (partial). 366 Table 19.17 Typical accident sequences and mitigation list (partial). 367 Index 2SEAS, 391 3D printing, 313, 332 Abaqus, 240, 247 accelerometer, 297 acceptance tests, 352, 358, 368 Access Hatches, 54 Accident List, 364 Accident Sequences and Mitigation, 366 ACIS, 191, 242 ACSYNT, 123 ADS, 123 aero-elasticity, 265, 297 aerocalc, 147 aerodynamics, 125 airframe, 214 codes, 125 lifting surfaces, 21 RANS, 228 simple wing theory, 33 ailerons, 22, 40, 80, 221, 269 air trafic, 353, 368 AirCONICS, 189 designing Decode-1, 192 example code for Decode-1, 399 structural modeling, 240 airfoil, 191 three-dimensional analysis, 210 two-dimensional analysis, 208 Airframe Load Tests, 290 airworthiness, 103, 349, 375 Anaconda, 147 Ancillaries, 88 ANSYS Fluent, 196, 200 AnyLogic, 125 Apache, 126 approach speed, 145, 149, 180, 376 approval, see Regulatory Approval Arduino, 86 aspect ratio, 21, 34, 162, 167, 170, 206, 266 Assembly Mechanisms, 54, 342 auto landing, see Flight Tests auto take-off, see Flight Tests autonomous light, 4, 357, 378 autopilot, 73, 84, 86 data capture, 368 failure, 108 radio channels, 357 testing, 378 Aviation Authority Requirements, 349 avionics, 73 diagram, 73 placement, 47 power supplies, 76 System Description, 356 testing, 300 trays, 50 batteries, 71, 383 bayonets, 345 beam theory, 239, 243 beyond line of sight, see BLOS blockage, see wind tunnels tests BLOS, 108, 359 bolts, conventional, 346 Boxer, 204 Breguet range equation, 125, 169 bulk modulus, 334 CAD Codes, 120 camera mountings, 51 carbon iber, 332 Cases, Storage and Transport, 347 cell height boundary layer, 203 Centaur, 204 Center of Gravity, see CoG CFD, 11, 119, 195 CFRP, 332 chopped strand, 340 chord, 177, 319 Clamps, 346 climb performance, 148, 167, 174, 224, 375 coeficient lap hinge moment, 221 of drag, 35, 151, 167, 202, 216, 285 of friction, 167 of lift, 33, 35, 41, 124, 149, 167, 176, 198, 202, 230, 268 of parasitic drag, 167, 232 of pitching moment, 35, 221, 223, 268 of roll, 223 of thrust, 174 of yaw, 223 tail volume, 221 CoG, 124, 176, 273, 310 control, 279 Estimation, 170 typical table, 273 UAS CoG light test, 377 compliance testing, 113 components, externally sourced, 4, 331 composites, 340 Computational Fluid Dynamics, see CFD constraint analysis, 144 the constraint space, 146 Constraints, 101 contingency planning, 371 weight, 278 control reversal, 265, 297 estimating onset speeds, 266 control surface, 22, 82, 262, 272, 290, 352, 382 failure, 108 inputs, 370 control system, 24, 73, 356 software management, 368 costs, 125 management, 114 covers, 37 cruise performance, 148 data-bases, 126 database, 352 databases, 363 decision making, 7 decision support, 123, 125 DECODE, 6 Decode-1 AirCONICS, 192 AirCONICS code, 399 analysis with Fluent, 228 analysis with XFLR5 Aerodynamics, 215 Control Surfaces, 221 Stability, 223 control surfaces, 221 detail design, 313 FEA, 245 Fluent, 228 ground vibration test, 297 lifting surfaces, 230 spar delections, 244 spar layout, 246 spreadsheet, 174 stability, 223 Vn diagram, 238 wind tunnel testing, 284 XFLR5, 215 Decode-2 spreadsheet, 177Index 443 design algorithm, 146 checklist, 117 concept, 8, 123 initial constraint analysis, 127 managing the process, 144 constraints, 129 computational implementation, 145 constraint analysis report, 146 detail, 11 Decode-1, 313 fuselage, 306 hand sketches, 311 master sketches, 311 wings, 318 drivers, 29 in-service and de-commissioning, 13 manufacturing, 12 preliminary, 10 aerodynamic and stability analysis, 195 geometry, 189 structural analysis, 237 requirements, 145 responsibility allocation, 112 the brief, 113, 127 the process of, 101 the stages of, 6 topology, 130 detail design, see design Digital DatCom, 229 dihedral, 191 divergence, 265, 297 estimating onset speeds, 266 Documentation, 349 drag induced, 42 Dutch roll, 223 electric motors see motors, 66 elevators, 22, 80, 139, 221, 269, 287 structure, 240 XFLR5, 200 Emergency Procedures, 360 empennage, 45 endurance, 15, 62, 72, 77, 89, 102, 129, 166, 355 engines control, 70 failure, 107 glow-plug IC, 62 IC Liquid Fueled, 59 mountings, 48 servicing, 382 spark ignition gasoline IC, 62 testing, 65 environment, 128 epoxy, 340 ESDU, 229 Euler-Bernoulli beam theory, 243 EVLOS, 359 Experimental Testing and Validation, 281 Expired Items Time and Flight, 382 extended visual line of sight, see EVLOS failure modes, 104 aerodynamic and control, 105 autopilot, 108 control surface, 108 effects, 362 engine, 107 motor, 107 primary Tx/Rx, 108 safety case, 350 structural, 106 FDM, 335 ABS, 335 FEA, 11, 245 analysis of 3D printed parts, 255 analysis of iber or Mylar clad foam parts, 255 complete spar and boom model, 250 model preparation, 246 Finite Element Analysis, see FEA laps, 22, 41, 221, 269 Flight Control Software, 383 light envelope, 369 Flight Planning Manual, 368 light simulators, 227444 Index light tests auto landing, 380 auto take-off, 380 Autonomous Flight Control, 378 Engine Failure, Idle and Throttle Change, 377 Fuel Consumption, 377 Operational and Safety Flight Scenarios, 381 safety, 361 UAS CoG (MANUAL mode), 377 UAS performance (MANUAL mode), 375 Fluent, see ANSYS Fluent lutter, 265, 297 estimating onset speeds, 266 foam, 337 cladding iber, 339 Mylar, 339 hot-wire cutting, 337 Fuel Systems, 70 fuel tanks, 24 integral, 44, 52 Fused Deposition Modeling, see FDM fuselage, 23, 45 detail design, 306 generators, 71 Geometry Codes, 120 glass iber, 340 glow-plug, see engines Goals, 101 GPS, 25 Gridgen, 204 ground control system, see control system ground vibration test, see structural testing gust load, 238 Hazards Operational, 363 health monitoring, 17, 90 hinge moment, 221 horseshoe vortex, 198 IGES, 191, 242 induced drag, see drag inertia stability, 226 structural dynamics, 265 XFLR5, 198 Instrumentation and recording of light test data, 370 interface deinitions, 112 iron bird, 342 ISA, 128 Javaprop, 68, 166 Jupyter, 145, 146 k-� , 205 k-� SST, 200 landing gear, 27, 191 Laser Cutting, 339 laser sintering see SLS, 332 Lessons Learned, 385 lifting line, 198 Lifting Surfaces, 21 LiPo see batteries, 71 locking pins, 345 Longitudinal Stability, 170 Maintenance, 381 Overall Airframe, 382 Record Keeping, 384 Schedule, 360 maneuver load, 238 Manufacture, 331 Manufacturing Methods, 5 Maximum Take-Off Weight, see MTOW Meshing, 204 mission, 128 Mission Planning, 125 mode shape, 269, 296 morphology, 7 motors brushless, 66, 77 control, 70 failure, 107 mountings, 48Index 445 MTOW, 15, 60, 146, 166, 247, 310 Mylar, 339 NACA airfoil, 197, 230, 320 nacelle structure, 45 NASA, 134, 196 natural frequency, 269, 296 OpenFoam, 196 OpenVSP, 123 Operational Simulation, 125 Operations Manual, 358 Brief Technical Description, 359 Maintenance Schedule, 360 Operating Limits, Conditions, and Control, 359 Operational and Emergency Procedures, 360 Operational Area and Flight Plans, 360 Organization, 359 Team Roles, 359 Oswald span eficiency, 153, 170 PaceLab, 123 panel method, 196, 198, 262 XFoil and XFLR5, 196 parasitic drag, 226 Parasolid, 191, 242 payload, 20, 27, 51 Payload Communications Systems, 87 phugoid, 223 pitch, 172 Pitot static, 87, 369, 384 Pixhawk, 87 Plane Maker, 228 Poisson’s ratio, 248, 334 polyester, 340 polyurethane, 337 Powering, 171 preliminary design, see design Primary Control Transmitter and Receivers, 73 Primary Tx/Rx Failure, 108 propeller, 20, 68 actuator disc, 193, 233 data, 166 diameter, 172, 177 sizing, 171 Propulsion, 24, 59 Python, 147, 190 radio links, 73, 87 range, 15, 89, 102, 129, 169 RANS, 196 Solvers – Fluent, 200 receiver, 24, 358 GPS, 90 primary control, 73 video, 53 Record Keeping Maintenance, 384 Regulatory Approval, 349, 368, 383 reliability, 65, 107, 377 requirements, see design requirements lowdown, 112 Resilience and Redundancy, 90 Rhinoceros CAD package, 190 ribs, 38 ring vortex, 198 Risk Assessment Process, 362 roll control, 40 roll damping, 223 rudders, 22, 139, 221, 269, 290 safety case, 361 Schrenk approximation, 240 screws, conventional, 346 sealing, components, 335 Selective Laser Sintering, see SLS sense and avoid, 27 servos, 78 testing, 302 shear modulus, 334 short period modes, 223 SIMPLE solution method, 200 simulators see light simulators SLS, 332 metal, 334 nylon, 334 sealing, 335 SolidWorks, 120, 303446 Index Spalart-Allmaras, 200, 205 spar delections, 244, 250 spars, 21, 37, 190, 221 FEA, 250 sizing, 169 testing, 293 spats, 95 spiral mode, 223 SPOTTER, 20 avionics diagram, 73 design brief, 184 engines, 64 fuel tank sensors, 71 geometry, 187 integral fuel tank, 53 nacelle, 46 payload pod, 51 spreadsheet, 182 wing, 37 Spreadsheet Based Concept Design, 165 stability derivatives, 224 stall, 34, 195, 281, 355 Star-CD, 196 static margin, 170 Steering, 95 STEP, 191, 242 structural analysis, 119, 125 codes, 125 preliminary, 237 using simple beam theory, 243 structural dynamics, 265 structural failure, 106 structural loading calculations, 169 structural mounting and loading, 293 structural testing dynamic, 296 instruments, 290 static, 294 structure internal, 23 SULSA, 51, 93 Suspension, 95 SVN, 126 sweep, 191, 319 synthesis, 7 System Description, 351 Airframe, 352 Flight Data, Acceptance, 358 Ground Control System, 356 Performance, 355 systems engineering, 110 tail, 45, 57 take-off, 68 auto, 380 performance, 148, 356 run, 375 taxonomy, 7 telemetry, 25, 88 Test Flight examples, 375 planning, 369 Tgrid, 204 Three-Dimensional Printing, see 3d printing thrust, 124, 172 thrust to weight ratio, 146, 154, 176 Tool Selection, 119 topologies, 133 TortoiseSVN, 126 transmitter, 358 payload, 87 primary control, 73 transponders, 27 TRIZ, 136 turbulence model, 196, 200 choice, 204 turn performance, 148 twist, 191, 319 UAVs A Brief Taxonomy, 15 morphology, 19 UIUC database, 59, 166, 198 undercarriage, 27, 93 attachment, 55 load test, 295 retractable wing housed, 42 retractable systems, 97Index 447 V-tail, 58, 139 validation, 281 value driven design, 6 viscous sublayer, 209 visual line of sight, see VLOS VLOS, 359 Vn diagram, 238, 352 weight, 125 control, 273 estimation, 170 management, 114 Wheels, 93 wind tunnels tests, 282 blockage effects, 284 calibrating the test, 284 elevator effectiveness, 287 mounting the model, 282 rudder effectiveness, 290 typical results, 287 wing, 33 attachment, 47 covers, 37 divergence, see divergence fuselage attachments, 38 loading, 146, 154, 177 morphing, 15, 40, 137 ribs, 38 span, 177, 319 tips, 42 Wiring Looms, 342 Wiring, Buses and Boards, 82 work-breakdown structure, 110 woven rovings, 340 X-Plane, 227 XFLR5, 195 XFoil, 195 y+, 203 Young’s modulus, 244, 334
كلمة سر فك الضغط : books-world.net The Unzip Password : books-world.net أتمنى أن تستفيدوا من محتوى الموضوع وأن ينال إعجابكم رابط من موقع عالم الكتب لتنزيل كتاب Small Unmanned Fixed-Wing Aircraft Design - A Practical Approach رابط مباشر لتنزيل كتاب Small Unmanned Fixed-Wing Aircraft Design - A Practical Approach 
|
|